Main Text
1 Introduction
Prunella vulgaris L. (P. vulgaris) is a perennial herbaceous plant species in the Labiatae family, which withers after the summer solstice, and has been used in China for thousands of years [1]. The secondary metabolites of P. vulgaris include mainly triterpenoids, phenolic acids, flavonoids, volatile oils, and polysaccharides [2]. Many studies have shown that these secondary metabolites have a wide range of biological activities, including anti-viral, anti-inflammatory, anti-tumour, anti-hypertensive and anti-hyperlipidaemic functions [3-5].
Plant hormones are organic compounds with physiological activity produced by plants. These kinds of compounds are indispensable for plant biological activity and occur in small quantities, but they are of great importance, especially in the regulation of various plant growth and development processes and environmental responses of plants and in the regulation of the accumulation of plant secondary metabolites. According to their molecular structures and physiological functions, plant hormones are usually divided into ethylene (ET), indoleacetic acid (IAA), cytokinins (CKs), gibberellins (GAs), abscisic acid (ABA), jasmonates (Jas), salicy acid (SA) and brassinosteroids (BRs). ET can promote the ripening of fruits, and its biosynthesis is related to IAA [6]. In addition, fruit ripening is regulated by the transcription factor ABA-stress-ripening (ASR), and ASR gene expression is affected not only by sucrose and ABA but also by JAs and IAA [7]. Moreover, IAA not only plays an important role in the ripening process of fruits during the early development and morphology of plants but also is relevant. Plants are often attacked by a wide range of microbial pathogens in their environment. Plant hormones such as SA, JAs, ABA and ET play a central role in the biological stress signalling associated with pathogen infection, mainly through synergy and antagonism, to regulate the protective response of plants to biotic and abiotic stress [8]. Studies have shown that CKs are purine-based plant growth regulators in plants whose signals can delay leaf senescence, promote bud branching, and specifically affect the growth of plant buds and roots [9]. Through the analysis of CK concentrations in plant tissues at different stages, the relationships between CK levels and the senescence process of various tissues and plant species have been revealed.
RA is a major component of phenolic acids in P. vulgaris and controls the quality of this medicinal plant according to National Chinese Pharmacopeia [10]. ABA could strongly enhance rosmarinic acid production [11].
In this study, we used reaction monitoring/multiple reaction monitoring (SRM/MRM) to quantitatively reveal the dynamic changes of phytohormone concentrations during P. vulgaris development. Furthermore, the effect of exogenous ABA on RA accumulation was verified.
2 Materials and methods
2.1 Plant materials and growth conditions
P. vulgaris plants were selected from the Pharmaceutical Plantation Garden of Hunan University of Traditional Chinese Medicine located in Shangsha, China (28°08’N and 112°54’E), which is approximately 88 m above sea level. The site has a subtropical monsoon climate. The annual precipitation is 1361.6 mm. The annual mean temperature is 17.2 °C, and the annual sunshine duration is 1529.3 h.
The samples were collected from 5 different developmental stages: the heading stage (stage 1), the flowering stage (stage 2), the green-fruit stage (stage 3), the turning stage (stage 4) and the ripening stage (stage 5) (Table 1).
Table 1 Sample information of P. vulgaris.
Stage | Morphology | Collection date |
---|---|---|
1 | The spikes grow from the apex of the stem, the tip of the ear is pointed and tail-shaped, and the veining is obvious. It is composed of several round calyx and bracts. Each round of bracts oppose 2 pieces and form a fan. | April 15 - May 3 |
2 | Prototype of the bracts petals, some low-end bracts lilac flowers grow from the tip 3 to the tip. | May 4 - May 11 |
3 | Spikes apex open, corolla off, there are 4 small green nuts. | May 12 - May 20 |
4 | Bracts gradually from green to red-brown from the bottom upward, but remain green cobs. | May 21 - Jun 1 |
5 | Complete conversion of the entire ear bracts reddish brown, brown nutlets inner spikes. | Jun 2 - Jun 16 |
2.2 Method validation
The samples were mixed in equal amounts to prepare QC samples, and QC samples were used at each interval for a certain number of experimental samples in the sample queue to detect and evaluate the stability and repeatability of the system. Twelve plant hormones at ten concentrations and fixed amounts of internal standards (2H5-IAA, 2H4-SA, 2H6-iP, cis-OPDA, 2H4-ACC, 2H5-tzR, 2H6-ABA, 2H4-tZ and 2H6-iPR, purchased from Olchemim Ltd., Olomouc, Czech Republic) were prepared to establish calibration curves by plotting the peak area ratio against the concentrations of plant hormone derivatives with quartic measurements. The limits of quantitation (LOQs) and limits of detection (LODs) were determined at concentrations for which the signal-to-noise (S/N) ratios were 10 and 3, respectively. Calibration curves were generated from the MRM signals obtained from standard solutions based on the ratio of the chromatographic peak area for each analyte to that of the corresponding internal standard.
2.3 Sample preparation
Plant materials were frozen in liquid nitrogen and stored at -80 °C. The samples were ground in liquid nitrogen, and 80 ± 5 mg was then placed in a 2 mL centrifuge tube. Fifty microlitres of internal standard solution was then added, followed by 1 mL of acetonitrile aqueous solution (1% FA). The mixture was then vortexed for 2 min (Vortexer: QL-866). Afterward, the samples were then extracted at 4 °C in the dark for 12 h, after which they were centrifuged at 14000 g for 10 min (refrigerated centrifuge: Xiangyi, H1650-W). In total, 800 μL of the supernatant was removed and dried under a stream of nitrogen and then added to 200 μL reconstituted aqueous acetonitrile (1:1, v/v). The samples were then centrifuged at 14000 g for 10 min, after which the supernatant was removed for analysis.
2.4 UHPLC-ESI-MS/MS analysis
Plant hormone analysis was performed via an ultra-high-performance liquid chromatography (UHPLC) system (Waters I-Class LC) [12]. Separation of the plant hormones was performed on an ACQUITY UPLC BEH C18 column (1.7 µm, 2.1 mm × 100 mm, Waters) at a flow rate of 400 μL/min at a 45 °C oven temperature. Mobile phases A and B consisted of 0.05% formic acid in water and 0.05% FA in acetonitrile, respectively. A gradient of 0-10 min of 2% to 98% B, 10-10.1 min of 98% to 2% B, and 11.1-13 min of 2% B was adopted. The injection volume was 4 µL. A 5500 QTRAP mass spectrometer (AB SCIEX) was used for mass spectrometry analysis in positive/negative ion mode. The 5500 QTRAP ESI ionization source conditions were as follows: source temperature, 500 °C; ion source gas 1 (gas 1), 45; ion source gas 2 (gas 2), 45; curtain gas (CUR), 30; and ion spray voltage floating (ISVF), -4500 V. MRM was employed in the ion pair for the detection of plant hormones.
2.5 ABA treatment
The experiment was modified on the basis of literature [13]. P. vulgaris at heading stage with similar growth performance, such as similar leaf sizes and cluster sizes, was chosen to randomly groups, including the control group, ABA low concentration group (1 μg/mL), ABA middle concentration group (5 μg/mL), and ABA high concentration group (10 μg/mL). P. vulgaris per pot was distributed in a randomized complete block design with 3 blocks for 3 biological replicates. First, the initial contents of RA in the leaves of P. vulgaris from each group were determined. Then, the positive and negative sides of leaves were evenly and gently smeared with different ABA concentrations. ABA treatment was applied one time per day with five days. Leaves were randomly sampled to determine the increasing rate of RA content on the sixth day. The formula for calculating the increasing rate of RA content was as bellow: The increase rate of RA content = (final content of RA - initial content of RA) × 100%/initial content of RA.
2.6 Statistical analysis
SPSS 25.0 software was used for statistical analysis, and comparisons between groups were performed by one-way variance analysis. The results are expressed as average ± standard deviation, and p < 0.05 was considered statistically significant.
3 Results
3.1 Method validation
QC samples were used to inspect the stability of the detection. The RSD% of each test object was less than 30%, indicating that the data were stable and reliable (Figure 1).
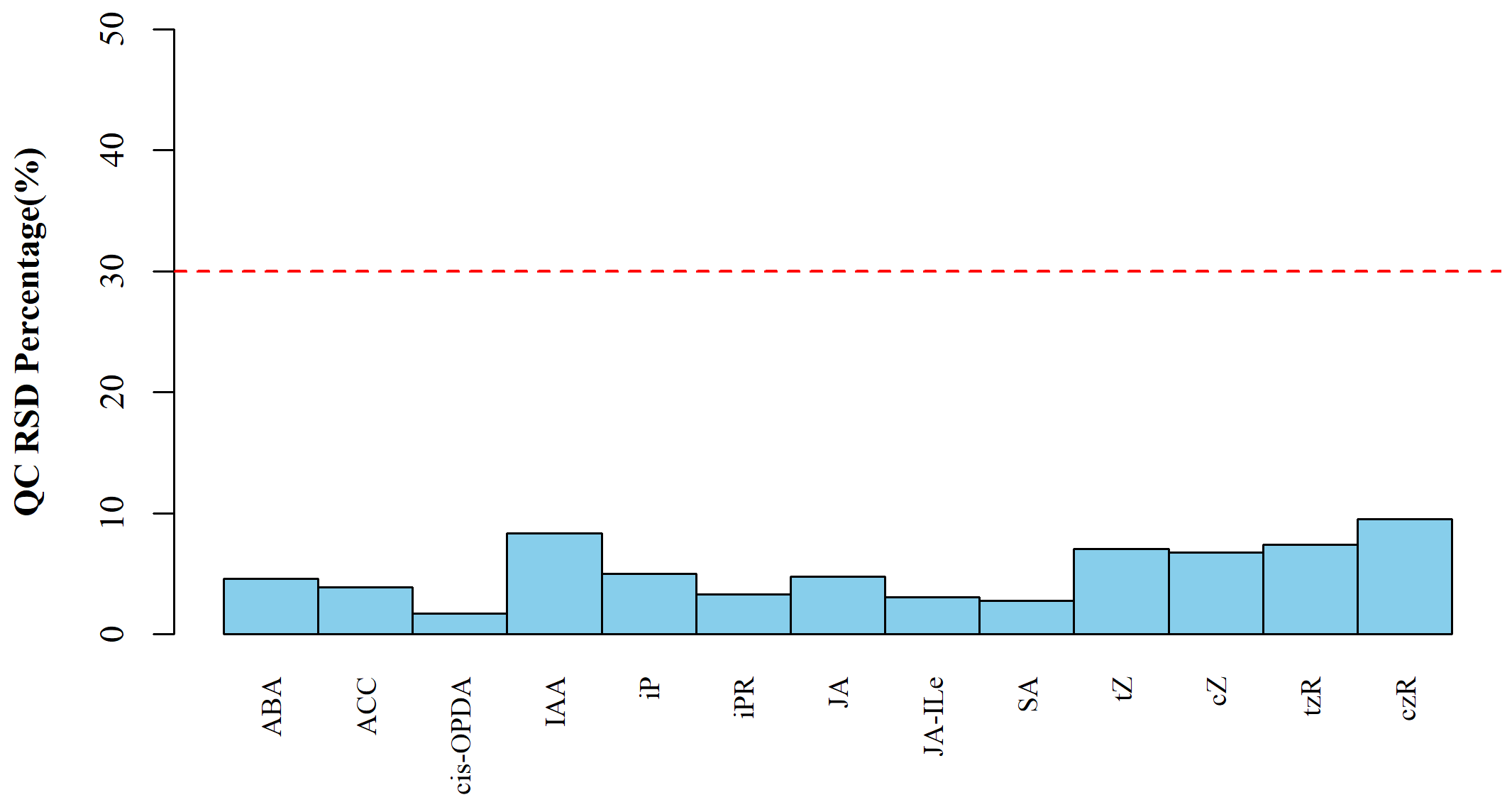
Figure 1 RSD distribution of QC.
Calibration curves were constructed on the basis of the peak area ratios of the analytes to the internal standards versus concentrations of the analyte standards. Calibration curves for the analysis of IAA, SA, iP, cis-OPDA, ACC, tzR, ABA, tZ, czR, JA-Ile, iPR, JAs and cZ were constructed by plotting the peak intensity ratio against the known analyte concentrations. Table 2 lists a summary of the calibration curves, correlation coefficients (R2 values), linear ranges, quantification limits (LOQs), and detection limits (LODs) of the 13 plant hormones. All 13 correlation coefficients were higher than 0.99. The LODs for the different plant hormones were in the range of 0.11-4.8 ng/mL, and the limits of quantification ranged from 1 to 10 ng/mL.
Table 2 Quantitation results of the 13 plant hormones under optimized UPLC-MS/MS conditions.
Phytohormone standard | Calibration curve | Correlation coeffificient (R2) | Linear range (ng/mL) | LOQ (ng/mL) | LOD (ng/mL) |
---|---|---|---|---|---|
IAA | y = 9.40331e-4x - 7.66121e-4 | 0.99919 | 2.5-100 | 2.5 | 0.46 |
SA | y = 0.02974x + 0.10929 | 0.99993 | 2.5-500 | 2.5 | 0.68 |
iP | y = 0.01311x - 0.00553 | 0.99919 | 1-500 | 1 | 0.25 |
cis-OPDA | y = 3187.47294x - 703.03646 | 0.99978 | 1-1000 | 1 | 0.25 |
ACC | y = 0.00488x + 0.02077 | 0.99948 | 10-500 | 10 | 4.8 |
tzR | y = 0.03845x - 0.01370 | 0.99958 | 1-500 | 1 | 0.18 |
ABA | y = 0.02667x + 0.15605 | 0.99988 | 1-500 | 1 | 0.25 |
tZ | y = 0.06376x - 0.00140 | 0.99965 | 1-100 | 1 | 0.25 |
czR | y = 0.03322x + 2.71542e-4 | 0.99916 | 2.5-500 | 2.5 | 0.50 |
JA-ILe | y = 5.44523e4x - 879.26344 | 0.99998 | 1-500 | 1 | 0.30 |
iPR | y = 5.44523e4 x - 879.26344 | 0.99923 | 1-50 | 1 | 0.22 |
JA | y = 4370.02946x + 23620.81526 | 0.99946 | 1-1000 | 1 | 0.43 |
cZ | y = 0.06489x + 0.00445 | 0.99714 | 1-100 | 1 | 0.11 |
3.2 Temporal distribution of plant hormones
During the development of P. vulgaris L., the changes in IAA concentration in the dissected spikes became apparent. From stage 1 to 3, the IAA concentration increased gradually from 14.19 ng/g to 869.12 ng/g, and there were significant differences (p < 0.05). Although it was maintained at a delicate dynamic balance between stage 3 and stage 4, the IAA concentration increased significantly from stage 4 to stage 5 and reached a peak value of 1766.33 ng/g (p < 0.05), and the level increased from stage 1 to stage 5 by 123.48-fold (Figure 2). The SA concentration from stage 1 to stage 2 increased slowly from 152.63 ng/g to 266.54 ng/g. Starting at the point at which the spikes turned brown, the SA concentration increased to 1228.53 ng/g (p < 0.01) until all the spikes were brownish red in colour, and the SA concentration peaked at 1843.17 ng/g (Figure 2). The increasing trend of cis-OPDA before stage 2 was not obvious. The cis-OPDA concentration increased significantly from 127.78 ng/g to 261.65 ng/g during stage 2 to stage 3 (p < 0.05). After stage 3, the upward trend slowed, and the change was not significant (p > 0.05). In addition, JA-Ile and JAs were also detected in the spikes of P. vulgaris L. during the five different growth periods, but no obvious trend was found (Figure 2). The ACC concentration peaked at 23.26 ng/g, which occurred before stage 3. As the spikes began to turn colour, the ACC concentration decreased to 11.56 ng/g (p < 0.01), but after stage 5, the ACC concentration did not change significantly (Figure 2). The ABA concentration was 10.00 ng/g at stage 1. It then remained low at stage 2, while it increased to 158.56 ng/g at stage 3 (p < 0.05). The ABA concentration decreased after stage 3, but the change was not significant (Figure 2). Six endogenous CKs, trans-zeatin (tZ), trans-zeatin riboside (tZR), cis-zeatin (cZ), cis-zeatin riboside (cZR), N6-(2-isopentenyl) adenine (iP), and N6-(2-isopentenyl) adenosine (iPR), were identified in the spikes of P. vulgaris L., whereas DHZ was not detected. The concentration of tzR was relatively stable before stage 3, with no significant change. However, its concentration decreased rapidly from 1.14 ng/g to 0.44 ng/g during stage 3 to stage 4 (p < 0.01). At stage 1, the concentrations of tZ and cZR were as high as 2.2 ng/g and 7.46 ng/g, respectively, but the concentrations decreased significantly at stage 2 to 0.96 ng/g and 4.96 ng/g, respectively (p < 0.05). From stage 2 to stage 3, the tZ concentration increased to 2.06 ng/g, and the czR concentration peaked at 13.31 ng/g (p < 0.01). The cZ concentration was below quantifiable values before stage 2, but its concentration was quantifiable thereafter, ranging from 0.06 ng/g to 3.41 ng/g from stage 3 to stage 5, respectively. The iP concentration remained at a low state before stage 2 but gradually increased from 0.33 ng/g at stage 2 to 1.17 ng/g at stage 5. The iPR concentration decreased from 0.54 ng/g to 0.31 ng/g during stage 1 to stage 2, respectively (p < 0.05) (Figure 2).
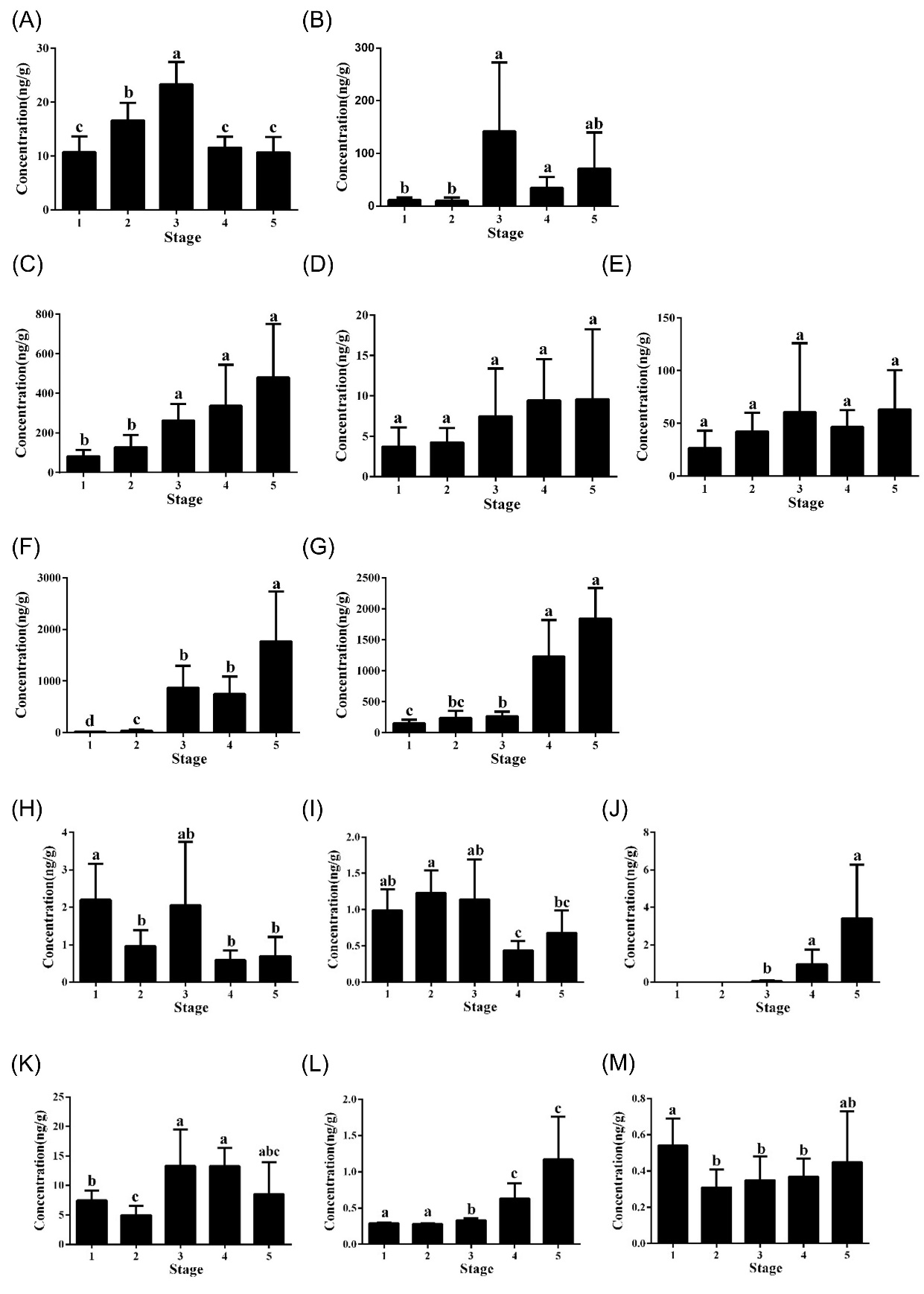
Figure 2 The concentrations of plant hormones in P. vulgaris during the development stages. (A) ACC: 1-aminocyclopropane-1-carboxylic acid; (B) ABA: abscisic acid; (C) cis-OPDA: cis(+)-12-oxophytodienoic acid; (D) JA-ILe: jasmonic acid-isoleucine; (E) JA: jasmonates; (F) IAA: indoleacetic acid; (G) SA: salicy acid; (H) tZ: trans-zeatin; (I) tZR: trans-zeatin riboside; (J) cZ: cis-zeatin; (K) cZR: cis-zeatin riboside; (L) iP: N6-(2-isopentenyl) adenine; (M) iPR: N6-(2-isopentenyl) adenosine. Columns with different lowercase letters were significantly different (p < 0.05, least significant difference test). Values are expressed as average ± standard deviation, n = 6.
3.3 Effect of ABA on the accumulation of RA
Secondary metabolites production in plants is regulated by several factors including environmental variables and plant growth regulators [14]. RA is an important secondary metabolite, commonly found in species of the Lamiaceae and Boraginaceae family. The biosynthesis of RA has been vastly investigated for two reasons: (1) RA has been shown to be a useful compound in the field of medicine, and as a food additive, (2) accumulated RA contributes to the defense against microbes [15].
ABA is a stress hormone that coordinates the complex networks of stress responses and its content is rapidly changed in response to stresses [15]. The ABA has been recognized as a stress hormone coordinating the complex networks of stress responses [16]. Several recent studies have showed that, changes in the amount of endogenous ABA may play an important role in the accumulation of RA and related phenolic compounds [17-23].
The effects of application of ABA (0, 1, 5, 10 μg/mL) to shoot leaves of P. vulgaris on RA content was evaluated (Figure 3). Five days after elicitation with ABA, an increase of RA content was observed, which was significantly enhanced with 10 μg/mL ABA (p < 0.05).
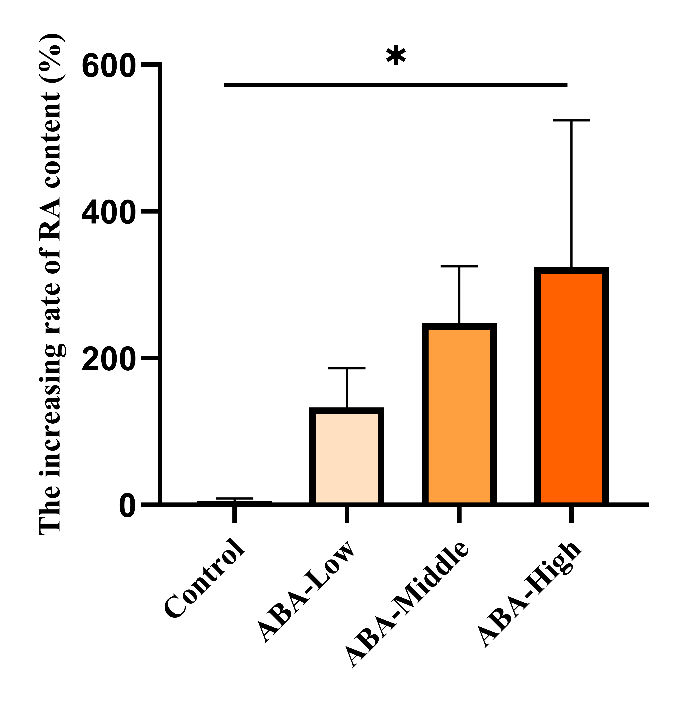
Figure 3 Effect of different concentrations of ABA on the increasing rate of RA content (average ± standard deviation, n = 3, compared with control, * p < 0.05).
4 Discussion
4.1 Analysis of changes in IAA concentrations
IAA participates in the morphological changes of P. vulgaris L. throughout its growth process. From stage 2 to stage 3, the IAA concentration increased rapidly, which is presumably related to P. vulgaris fruit formation in the spikes. As the spikes matured, the IAA concentration reached its highest value. IAA has been reported to be found mainly in rice spikes [24]. A high IAA accumulation in spikes is associated with auxin biosynthesis, metabolic processes, polar auxin transport, and auxin-mediated signalling [25]. Moreover, it has been shown that high concentrations of IAA can promote ethylene biosynthesis by inducing ACC synthase (ACS) to stimulate the synthesis of ACC, thus promoting fruit ripening [6]. This may be the reason for the increase in IAA concentration after fruit formation in the green-fruit stage. At the same time, it also promotes a rapid increase in concentrations of ACC, the direct precursor of ethylene. ACC, the direct precursor of ET, is subsequently used in large quantities for ethylene synthesis. Overall, auxin is considered to be an essential plant growth promoter and a determinant of plant morphogenesis [26,27].
4.2 Analysis of changes in SA concentrations
SA is an important mediator of the plant defence response to pathogens; this hormone can activate pathogen-associated molecular pattern (PAMP)-triggered immunity (PTI) and effector-triggered immunity (ETI), mediates systemic-acquired resistance (SAR) and is a key defence signalling molecule against biotrophs [28]. JAs are produced during the defence response against necrotrophic pathogens [29]. In general, these two hormone pathways function antagonistically. SA usually antagonizes JA signalling through processes that depend on Non-expressor of pathogenesis-related genes 1 (NPR1), suppressor of SA insensitivity 2 (SSI2), WRKY transcription factors and MPK4. Although antagonism between SA and JA is bidirectional, the main regulatory activity appears to be repression of JA signalling via SA-dependent cues. Microarray studies using different SA- and JA-signalling mutants have identified considerably more JA-dependent genes repressed by SA signalling than SA-dependent genes repressed by JA signalling [30].
In our study, the SA concentration was relatively low before the turning stage, and as the spikes turned brown, the SA concentration increased rapidly. Studies have shown that SA collects at sites of infection during the defence process of plants [31]. It is speculated that, as P. vulgaris spikes begin to brown, pathogens invade the site of infection, the immune function P. vulgaris is induced, and the SA concentration dramatically increases in the spikes. Our study also found that, at stage 5, the JA concentration in the spikes did not change significantly and were maintained at low levels.
4.3 Analysis of changes in ABA concentrations
ABA is considered a negative regulator of disease resistance. This is due to the negative activity of ABA interference on the regulatory activity of SA, JA and ET in response to biological stress, as well as additional ABA on common components of stress signals [32]. ABA may indirectly affect SA signalling through its influence on JA signalling, and vice versa. Cross-talk between SA and ABA is bidirectional [30]. Moreover, high concentrations of ABA inhibit the biosynthesis of ET [33]. In this study, the ABA concentration reached its peak during the green-fruit stage. On the one hand, ABA is related to the formation of fruits, and ABA can promote fruit development; on the other hand, the increase in ABA concentration is associated with susceptibility to infection during that time [34,35]. After the spikes begin to turn colour, their ABA concentration decreases rapidly, which may be related to the antagonistic effect of the sharp increase in SA concentration after infection. At this stage, the ACC concentration decreases rapidly, and ET begins to be synthesized to promote fruit maturity.
The ABA concentration decreased rapidly and may also be related to high levels of IAA. IAA inhibits the expression of the ABA biosynthesis gene NCED and the ABA receptor gene PYR but promotes the expression of the ABA degradation gene CYP707A, which leads to a reduction in ABA content. ABA also inhibits IAA accumulation genes, auxin transporter genes (FaPIN), auxin synthesis pathway genes and flavin monooxygenase genes (FaYUCCA). This may explain why the IAA concentration does not increase but instead decreases during the turning stage [36].
4.4 Analysis of changes in CK concentrations
CKs interact with light signals involved in numerous physiological processes, such as the induction of flowering and leaf expansion [37]. In addition, CKs can slow these senescence-accompanying changes. Among these CKs, tZ clearly had the highest antisenescence activity, while cZ and iP were considered CKs with low or no antisenescence effects [38]. We found that tZ, tZR and iPR presented high contents in the early stage. For example, tZ had the highest concentration in the heading stage, and the tZR concentration remained at a high state before the green-fruit stage. This may be related to the growth and differentiation of the cambium. Moreover, plant leaf senescence is directly related to decreases in CK concentration and activity [39]. Leaf senescence is the last step in leaf development and is usually accompanied by a change in colour from green to brown or yellow [40]. Yellowing not only depends on age but also can be caused by many other factors, such as harvest and environmental stress [41]. During the late stage of P. vulgaris growth, concentrations of both tZ and tZR decrease, which may be related to fruit ripening and spike senescence. The concentration of the two is inversely proportional to the ripening process of P. vulgaris. Studies have shown that the effects of CKs are often affected by interactions with other hormones, such as auxin [42]. IAA inhibits the biosynthesis of tZ through the isopentenyladenosine-5’-monophosphate ip nucleotide-independent pathway [43]. The degradation of CKs is affected by downregulating the expression of CKX2, CKX4 and CKX7 genes and upregulating that of CKX1 and CKX6 [44]. Increased CK levels lead to a rapid increase in auxin biosynthesis in seedlings, developing roots and leaf tissues [45,46]. The concentrations of cZ, cZR and iP increased significantly in the green-fruit stage, and the concentrations of cZ and iP increased from the green-fruit stage to the ripening stage. It is speculated that these hormones are present mainly in gradually maturing fruit.
4.5 Effects of hormone levels on secondary metabolites
The secondary metabolites of P. vulgaris have many pharmacological activities. Plant hormones can affect the synthesis and accumulation of secondary metabolites by activating or inhibiting the activity of corresponding transcription factors that regulate the expression of key enzyme genes related to plant secondary metabolism. Among these hormones, IAA, ABA, SA and CKs can affect the shikimate/phenylpropanoid pathways, which are involved in the biochemical synthesis of phenolic compounds (Figure 4). IAA can induce the accumulation of flavonoids by regulating the activities of L-phenylalanine ammonia-lyase (PAL) and chalcone synthase (CHS) [47]. In addition, studies have shown that auxin can induce the biosynthesis of rutin [48]. ABA, SA and CKs can increase the content of phenols and flavonoids by enhancing the activity of PAL or inducing its expression. SA promotes the accumulation of soluble phenols and lignin, and CKs promote the accumulation of anthocyanins [19,49,50]. In addition, cytokinin-SHY2 signalling can regulate the content of flavonoids in the roots [51]. R2R3-MYB, bHLH, and WD40 proteins in the JA signalling pathway are involved in regulating the biosynthesis of anthocyanin. The synthesis of most plant anthocyanins occurs via the R2R3-MYB transcription factor or the MYB-bHLH-WD40 complex (MBW). Among these transcription factors, MYBL2 and CAPRICE (CPC) of the R2R3-MYB complex negatively regulate the biosynthesis of anthocyanins by hindering the formation of the MBW complex, inhibiting the transcriptional activation activity of the complex, and therefore inhibiting the expression of anthocyanin synthesis genes [52].
Studies have shown that ABA and JAs can promote the biosynthesis of terpenoids. The WRKY and bHLH family transcription factors in the JA signalling pathway are involved in the regulation of the biosynthesis of tanshinone, salvianolic acid and paclitaxel. These transcription factors negatively regulate the biosynthesis of tanshinone and salvianolic acid and positively regulate Taxol biosynthesis [53-56] (Figure 4).
Auxin can quickly downregulate the expression of genes encoding essential indole alkaloid biosynthesis enzymes (the strictosidine synthase (SSS) gene and the tryptophan decarboxylase (TDC) gene) [57]. All the transcription factors of the bHLH, AP2/ERF and WRKY families in the JA signalling pathway are involved in the regulation of the biosynthesis of monoterpenoid indole alkaloids (MIAs), vinblastine, a variety of nicotine alkaloids, isoquinoline alkaloids and berberine [58]. Moreover, SA has a cumulative effect on alkaloids but has an inhibitory effect on the synthesis of alkaloids induced by JA [59] (Figure 4).
In addition, indole-3-acetaldoxime (IAOx) represents the junction between the core pathway of glucosinolates (GLSs) and the auxin synthesis pathway. IAOx plays a role in the regulating branch points in the dynamic balance between the indole glucosinolate core pathway and the auxin synthesis pathway. JAs, SA, ET and ABA can induce GLS biosynthesis genes and increase GLS concentrations [60]. ABA can induce the biosynthesis of isoriccardin C, marchantin C and riccardin F, which are classified as bis(phenylbenzyl) bisbibenzyls [61] (Figure 4).
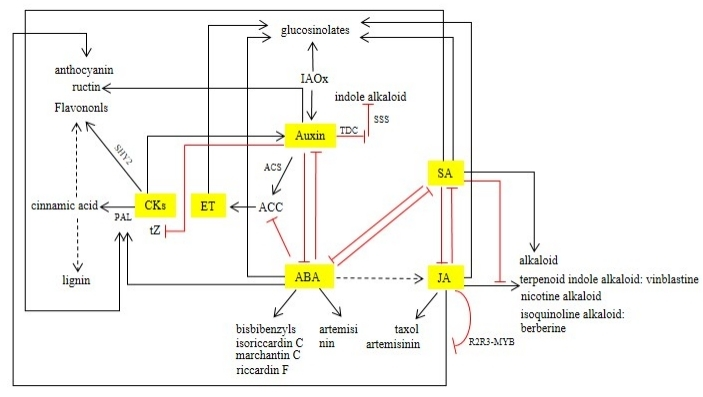
Figure 4 Summary of the results of this study. Interactions of plant hormones and their effects on secondary metabolites. → Forward regulation; —| Reverse regulation.
According to the results of this study, ABA treatment of 10 μg/mL has increased RA content significantly as compared to non-treated group. Similar results have recently been reported by other researchers in Melissa officinalis [14] and Dracocephalum moldavica L. [62].
ABA-induced increase in RA content could be in part due to the role of ABA in enhancing PAL activity and over-expression of genes involved in RA biosynthesis pathways [63,64]. Cui et al. reported that the activities of PAL and TAT and consequently accumulationof the phenolic compounds including RA, salvianolic acid and caffffeic acid are signifificantly increased by ABA treatment [65]. Shen et al. found that ABA up-regulates the expressions of PAL, TAT and RA synthase (RAS) genes [64]. Weiss and Ori found that PAL activity is suppressed with exogenous ABA treatment [66]. PAL activity in dragonhead leaves is increased by exogenous ABA treatment of 5–40 μM. Increased PAL activity with an increase in ABA levels was also reported in Fragaria ananassa [67], Cynomorium songaricum [65] and Microdochium nivale [19].
5 Conclusion
In this study, the MRM method was used to quantitatively analyse various plant endogenous hormones in P. vulgaris during development, revealing the dynamic changes in hormones and the relationships between hormones and the phenotypic changes, growth and development of P. vulgaris. We confirmed that exogenous ABA could significantly promote the accumulation of RA in P. vulgaris. The regulatory activity of plant hormones on the accumulation of secondary metabolites can serve as a basis for elucidating the growth and development mechanism of P. vulgaris in the future and provides a reference for studying the molecular mechanism through which plant hormones regulate secondary metabolites.
Back Matter
Acknowledgments
Not applicable.
Conflicts of Interest
The authors declare that they have no conflict of interest.
Author Contributions
Q.S., Z.Z., Y.Lin, Y.Li, and J.X. performed the experiments. Q.S. and Z.Z. analyzed the data, wrote this manuscript. B.X., L.L. and P.W. revised this manuscript. L.L. designed and supervised this research. All authors approved the final version of this article, including the author list.
Ethics Approval and Consent to Participate
No ethical approval was required for this article.
Funding
This work was supported by "Hunan Province Science and Technology Innovation Leading Talent Project" (Grant No: 2021RC4034) and "Hunan Science and Technology Innovation Team Project" (Grant No: 2021RC4064).
Availability of Data and Materials
Data will be made available on request.
Supplementary Materials
Not applicable.
References
- Zhang Z, Xia B, Li Y, et al. Comparative proteomic analysis of Prunella vulgaris L. spica ripening. Journal of Proteomics 2021; 232: 104028.
- Bai Y, Xia B, Xie W, et al. Phytochemistry and pharmacological activities of the genus Prunella. Food Chemistry 2016; 204: 483-496.
- Li BY, Hu Y, Li J, et al. Ursolic acid from Prunella vulgaris L. efficiently inhibits IHNV infection in vitro and in vivo. Virus Research 2019; 273: 197741.
- Zheng XQ, Song LX, Han ZZ, et al. Pentacyclic triterpenoids from spikes of Prunella vulgaris L. with thyroid tumour cell cytostatic bioactivities. Natural Product Research 2022; 18: 1-9.
- Zhang Z, Zhou Y, Lin Y, et al. GC-MS-based metabolomics research on the anti-hyperlipidaemic activity of Prunella vulgaris L. polysaccharides. International Journal of Biological Macromolecules 2020; 159: 461-473.
- Pan L, Zeng W, Niu L, et al. PpYUC11, a strong candidate gene for the stony hard phenotype in peach (Prunus persica L. Batsch), participates in IAA biosynthesis during fruit ripening. Journal of Experimental Botany 2015; 66(22): 7031-7044.
- Jia HF, Chai YM, Li CL, et al. Abscisic acid plays an important role in the regulation of strawberry fruit ripening. Plant Physiology 2011; 157(1): 188-199.
- Fujita M, Fujita Y, Noutoshi Y, et al. Crosstalk between abiotic and biotic stress responses: a current view from the points of convergence in the stress signaling networks. Current Opinion in Plant Biology 2006; 9(4): 436-442.
- Kim HJ, Ryu H, Hong SH, et al. Cytokinin-mediated control of leaf longevity by AHK3 through phosphorylation of ARR2 in Arabidopsis. Proceedings of the National Academy of Sciences of the United States of America 2006; 103(3): 814-819.
- National Pharmacopoeia Committee. Chinese Pharmacopoeia; Chemical Industry Press: Beijing, China, 2020.
- Trócsányi E, György Z, Zámboriné-Németh É. New insights into rosmarinic acid biosynthesis based on molecular studies. Current Plant Biology 2020; 23: 100162.
- Kim J, Lee JG, Hong Y, et al. Analysis of eight phytohormone concentrations, expression levels of ABA biosynthesis genes, and ripening-related transcription factors during fruit development in strawberry. Journal of Plant Physiology 2019; 239: 52-60.
- Tong Q, Liu L, Zhao Y, et al. Transcriptome Remodeling in Response to Leaf Removal and Exogenous Abscisic Acid in Berries of Grapevine (Vitis vinifera L.) Fruit Cuttings. Horticulturae 2022; 8(10): 905.
- Burkart S, Manderscheid R, Wittich KP, et al. Elevated CO2 effects on canopy and soil water flux parameters measured using a large chamber in crops grown with free-air CO2 enrichment. Plant Biology 2011; 13(2): 258-269.
- Mousavi SM, Shabani L. Rosmarinic acid accumulation in Melissa officinalis shoot cultures is mediated by ABA. Biologia Plantarum 2019; 63: 418-424.
- Zhang J, Jia W, Yang J, et al. Role of ABA in integrating plant responses to drought and salt stresses. Field Crops Research 2006; 97(1): 111-119.
- Gagné S, Cluzet S, Mérillon JM, et al. ABA initiates anthocyanin production in grape cell cultures. Journal of Plant Growth Regulation 2011; 30(1): 1-10.
- Hao G, Ji H, Li Y, et al. Exogenous ABA and polyamines enhanced salvianolic acids contents in hairy root cultures of Salvia miltiorrhiza Bge. f. alba. Plant Omics 2012; 5(5): 446-452.
- Ibrahim MH, Jaafar HZ. Abscisic acid induced changes in production of primary and secondary metabolites, photosynthetic capacity, antioxidant capability, antioxidant enzymes and lipoxygenase inhibitory activity of Orthosiphon stamineus Benth. Molecules 2013; 18(7): 7957-7976.
- Liang Z, Ma Y, Xu T, et al. Effects of abscisic acid, gibberellin, ethylene and their interactions on production of phenolic acids in salvia miltiorrhiza bunge hairy roots. PLoS One 2013; 8(9): e72806.
- Villalobos-González L, Peña-Neira A, Ibáñez F, et al. Long-term effects of abscisic acid (ABA) on the grape berry phenylpropanoid pathway: Gene expression and metabolite content. Plant Physiology and Biochemistry 2016; 105: 213-223.
- Murcia G, Fontana A, Pontin M, et al. ABA and GA3 regulate the synthesis of primary and secondary metabolites related to alleviation from biotic and abiotic stresses in grapevine. Phytochemistry 2017; 135: 34-52.
- Flores G, Blanch GP, del Castillo MLR. Abscisic acid treated olive seeds as a natural source of bioactive compounds. LWT 2018; 90: 556-561.
- Cai WJ, Ye TT, Wang Q, et al. A rapid approach to investigate spatiotemporal distribution of phytohormones in rice. Plant Methods 2016; 12: 47.
- Xue LJ, Zhang JJ, Xue HW. Genome-wide analysis of the complex transcriptional networks of rice developing seeds. PLoS One 2012; 7(2): e31081.
- Benková E, Ivanchenko MG, Friml J, et al. A morphogenetic trigger: is there an emerging concept in plant developmental biology? Trends in Plant Science 2009; 14(4): 189-193.
- Enders TA, Strader LC. Auxin activity: Past, present, and future. American Journal of Botany 2015; 102(2): 180-196.
- Li N, Han X, Feng D, et al. Signaling Crosstalk between Salicylic Acid and Ethylene/Jasmonate in Plant Defense: Do We Understand What They Are Whispering? International Journal of Molecular Sciences 2019; 20(3): 671.
- Bürger M, Chory J. Stressed Out About Hormones: How Plants Orchestrate Immunity. Cell Host & Microbe 2019; 26(2): 163-172.
- Vlot AC, Dempsey DA, Klessig DF. Salicylic Acid, a multifaceted hormone to combat disease. Annual Review of Phytopathology 2009; 47: 177-206.
- Métraux JP, Signer H, Ryals J, et al. Increase in salicylic Acid at the onset of systemic acquired resistance in cucumber. Science 1990; 250(4983): 1004-1006.
- Mauch-Mani B, Mauch F. The role of abscisic acid in plant-pathogen interactions. Current Opinion in Plant Biology 2005; 8(4): 409-414.
- LeNoble ME, Spollen WG, Sharp RE. Maintenance of shoot growth by endogenous ABA: genetic assessment of the involvement of ethylene suppression. Journal of Experimental Botany 2004; 55(395): 237-245.
- Audenaert K, De Meyer GB, Höfte MM. Abscisic acid determines basal susceptibility of tomato to Botrytis cinerea and suppresses salicylic acid-dependent signaling mechanisms. Plant Physiology 2002; 128(2): 491-501.
- Thaler JS, Bostock RM. Interactions between abscisic‐acid‐mediated responses and plant resistance to pathogens and insects. Ecology 2004; 85(1): 48-58.
- Jia H, Jiu S, Zhang C, et al. Abscisic acid and sucrose regulate tomato and strawberry fruit ripening through the abscisic acid-stress-ripening transcription factor. Plant Biotechnology Journal 2016; 14(10): 2045-2065.
- Reeves I, Emery RJ. Seasonal patterns of cytokinins and microclimate and the mediation of gas exchange among canopy layers of mature Acer saccharum trees. Tree Physiology 2007; 27(11): 1635-1645.
- Hönig M, Plíhalová L, Husičková A, et al. Role of Cytokinins in Senescence, Antioxidant Defence and Photosynthesis. International Journal of Molecular Sciences 2018; 19(12): 4045.
- Hallmark HT, Rashotte AM. Cytokinin isopentenyladenine and its glucoside isopentenyladenine-9G delay leaf senescence through activation of cytokinin-associated genes. Plant Direct 2020; 4(12): e00292.
- Koyama T. A hidden link between leaf development and senescence. Plant Science 2018; 276: 105-110.
- Toscano S, Trivellini A, Ferrante A, et al. Physiological mechanisms for delaying the leaf yellowing of potted geranium plants. Scientia Horticulturae 2018; 242: 146-154.
- Mok DWS, Mok MC. Cytokinin metabolism and action. Annual Review of Plant Physiology and Plant Molecular Biology 2001; 52: 89-118.
- Nordström A, Tarkowski P, Tarkowska D, et al. Auxin regulation of cytokinin biosynthesis in Arabidopsis thaliana: a factor of potential importance for auxin-cytokinin-regulated development. Proceedings of the National Academy of Sciences of the United States of America 2004; 101(21): 8039-8044.
- Werner T, Köllmer I, Bartrina I, et al. New insights into the biology of cytokinin degradation. Plant Biology 2006: 8(3): 371-381.
- Bielach A, Hrtyan M, Tognetti VB. Plants under Stress: Involvement of Auxin and Cytokinin. International Journal of Molecular Sciences 2017; 18(7): 1427.
- Jones B, Gunnerås SA, Petersson SV, et al. Cytokinin regulation of auxin synthesis in Arabidopsis involves a homeostatic feedback loop regulated via auxin and cytokinin signal transduction. Plant Cell 2010; 22(9): 2956-2969.
- Kučerová D, Kollárová K, Vatehová Z, et al. Interaction of galactoglucomannan oligosaccharides with auxin involves changes in flavonoid accumulation. Plant Physiology and Biochemistry 2016; 98: 155-161.
- Lee Y, Lee DE, Lee HS, et al. Influence of auxins, cytokinins, and nitrogen on production of rutin from callus and adventitious roots of the white mulberry tree (Morus alba L.). Plant Cell Tissue and Organ Culture (PCTOC) 2011; 105(1): 9-19.
- Deikman J, Hammer PE. Induction of Anthocyanin Accumulation by Cytokinins in Arabidopsis thaliana. Plant Physiology 1995,108(1):47-57.
- Misra N, Misra R, Mariam A, et al. Salicylic acid alters antioxidant and phenolics metabolism in Catharanthus roseus grown under salinity stress. African Journal of Traditional, Complementary and Alternative Medicines (AJTCAM) 2014; 11(5): 118-125.
- Silva-Navas J, Moreno-Risueno MA, Manzano C, et al. Flavonols Mediate Root Phototropism and Growth through Regulation of Proliferation-to-Differentiation Transition. Plant Cell 2016; 28(6): 1372-1387.
- Zhao J, Chen L, Ma A, et al. R3-MYB transcription factor LcMYBx from Litchi chinensis negatively regulates anthocyanin biosynthesis by ectopic expression in tobacco. Gene 2022; 812: 146105.
- Cusido RM, Onrubia M, Sabater-Jara AB, et al. A rational approach to improving the biotechnological production of taxanes in plant cell cultures of Taxus spp. Biotechnology Advances 2014; 32(6): 1157-1167.
- Xia J, Ma YJ, Wang Y, et al. Deciphering transcriptome profiles of tetraploid Artemisia annua plants with high artemisinin content. Plant Physiology and Biochemistry 2018; 130: 112-126.
- Lenka SK, Nims NE, Vongpaseuth K, et al. Jasmonate-responsive expression of paclitaxel biosynthesis genes in Taxus cuspidata cultured cells is negatively regulated by the bHLH transcription factors TcJAMYC1, TcJAMYC2, and TcJAMYC4. Frontiers in Plant Science 2015; 6: 115.
- Zhang F, Fu X, Lv Z, et al. A basic leucine zipper transcription factor, AabZIP1, connects abscisic acid signaling with artemisinin biosynthesis in Artemisia annua. Molecular Plant 2015; 8(1): 163-175.
- Pasquali G, Goddijn OJ, de Waal A, et al. Coordinated regulation of two indole alkaloid biosynthetic genes from Catharanthus roseus by auxin and elicitors. Plant Molecular Biology 1992; 18(6): 1121-1131.
- Zhou M, Memelink J. Jasmonate-responsive transcription factors regulating plant secondary metabolism. Biotechnology Advances 2016; 34(4): 441-449.
- Zayed R, Wink M. Induction of pyridine alkaloid formation in transformed root cultures of Nicotiana tabacum. Zeitschrift fur Naturforschung - Section C Journal of Biosciences 2009; 64(11-12): 869-874.
- Malka SK, Cheng Y. Possible Interactions between the Biosynthetic Pathways of Indole Glucosinolate and Auxin. Frontiers in Plant Science 2017; 8: 2131.
- Kageyama A, Ishizaki K, Kohchi T, et al. Abscisic acid induces biosynthesis of bisbibenzyls and tolerance to UV-C in the liverwort Marchantia polymorpha. Phytochemistry 2015; 117: 547-553.
- Khaleghnezhad V, Yousefi AR, Tavakoli A, et al. Interactive effects of abscisic acid and temperature on rosmarinic acid, total phenolic compounds, anthocyanin, carotenoid and flavonoid content of dragonhead (Dracocephalum moldavica L.). Scientia Horticulturae 2019; 250: 302-309.
- Xiao Y, Zhang L, Gao S, et al. The c4h, tat, hppr and hppd genes prompted engineering of rosmarinic acid biosynthetic pathway in Salvia miltiorrhiza hairy root cultures. PLoS One 2011; 6(12): e29713.
- Shen L, Ren J, Jin W, et al. Role of NO signal in ABA-induced phenolic acids accumulation in Salvia miltiorrhiza hairy roots. Sheng Wu Gong Cheng Xue Bao 2016; 32(2): 222-230.
- Cui B, Liang Z, Liu Y, et al. Effects of ABA and its biosynthetic inhibitor fluridone on accumulation of penolic acids and activity of PAL and TAT in hairy root of Salvia miltiorrhiza. Zhongguo Zhong Yao Za Zhi 2012; 37(6): 754-759.
- Weiss D, Ori N. Mechanisms of cross talk between gibberellin and other hormones. Plant Physiology 2007; 144(3): 1240-1246.
- Jiang Y, Joyce DC. ABA effects on ethylene production, PAL activity, anthocyanin and phenolic contents of strawberry fruit. Plant Growth Regulation 2003; 39(2): 171-174.